The Physics of Muon Colliders
Novel technology and perhaps physics awaits us if we’re brave enough to build one.
Last time we contrasted the performance of lepton and hadron colliders. Hadrons are messy, composite things like protons that enjoy a relatively large mass. Leptons are tiny by comparison, but elementary. They are things like electrons and their antiparticle partners, positrons.
Protons versus Electrons
In a collider ring, protons can be spun up much faster because their internal mass inhibits the radiation of light: that “braking radiation” associated to centripetal acceleration. Electrons, with a mass almost 2000 times smaller, shine like high beams on a dark highway. All that light depletes their kinetic energy. They just can’t make it up to speed.
Higher collision velocity is crucial for probing particle physics, where the shrapnel from the explosion often includes less commonly seen particles like the Higgs Boson. That is why the Large Hadron Collider (LHC) uses protons to look for new physics.
The trade off leptons offer is cleanliness. Protons are clunky and full of subnuclear junk. The collisions are too. Lepton collisions are almost surgically clean by comparison.
Because of this, lepton colliders are used at lower energies to study the properties of particles with high precision. For example, SLAC used the SLC to tabulate the physics around the Z-boson and its decays.
To date, lepton colliders have typically meant two beams: one of electrons and one of positrons. At low energies, the two particles annihilate into a pair of gamma rays. As the energy ramps up, all kinds of things can come out.
Last time we advocated for the construction of a muon collider. Muons are 206 times heavier than the electron - and can therefore be accelerated much faster. They don’t radiate light nearly as much as the electron. Unlike the proton, the muon is an elementary particle, so a muon-antimuon collider can offer the best of both options.
The cost is one tough logistical hurdle: the muon is unstable. It typically decays in a few microseconds1.
This means a muon collider must create, accelerate and collide muons from scratch, all in a fraction of a second.
Today we’ll describe in detail how a muon collider works, and why it’s not so crazy to use them to search for new physics, particular around the Higgs boson.
About the Muon
The muon is a big fat electron. It has the same electric charge and the same generic features of the electron, except that it’s bigger. Thanks to the persistent impingement of cosmic rays from outer space, muons a common particle to find here on Earth. We’ve discussed the muon at length both in our Field Guide to Particle Physics, and a special miniseries on Cosmic Rays2.
A sizable chunk of your annual exposure to ionizing radiation comes from muons blasting through you from the upper atmosphere.
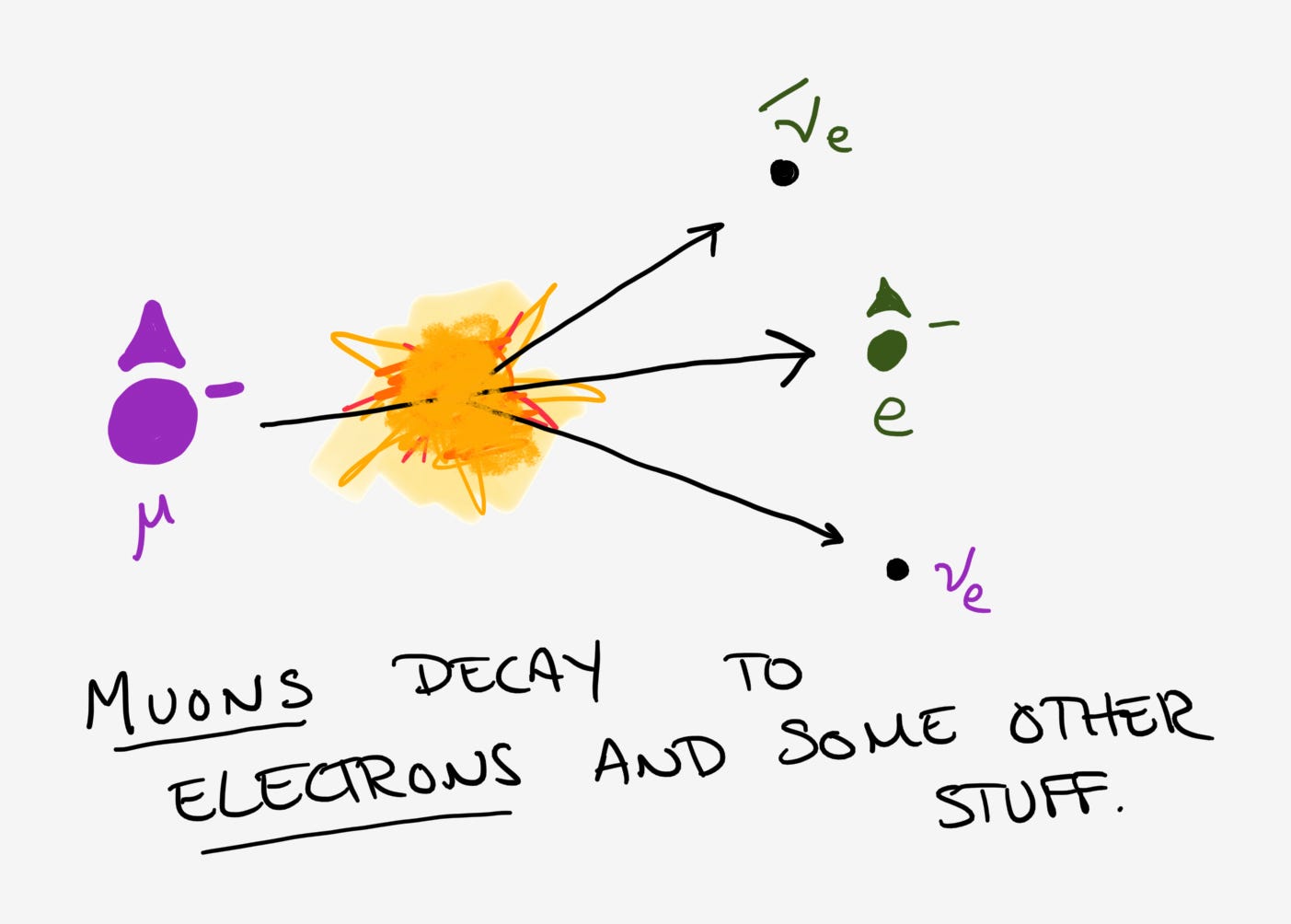
The muon only decays to the electron and other junk, typically3 a pair of neutrini. This restriction is mostly about its mass. At 105.7 MeV, the muon just isn’t heavy enough to decay into anything else; there’s nothing else for it to decay into.
This dearth of options is partly why the muon sticks around for much longer than other unstable particles, and this is precisely what makes it an excellent candidate for a collider beam. While 2.2 microseconds might not seem long, it’s an eternity by particle physics standards.
What’s amusing about those so-called cosmogenic muons - borne from atmospheric collisions with cosmic rays - is that they actually take much longer than 2.2 microseconds to reach us. At least from our perspective. Light can’t even make it that far in such a short a timeframe. Those muons are traveling very close to the speed of light, so close, in fact that Einstein’s theory of special relativity extends their lifetime by more than enough time to reach sea level4.
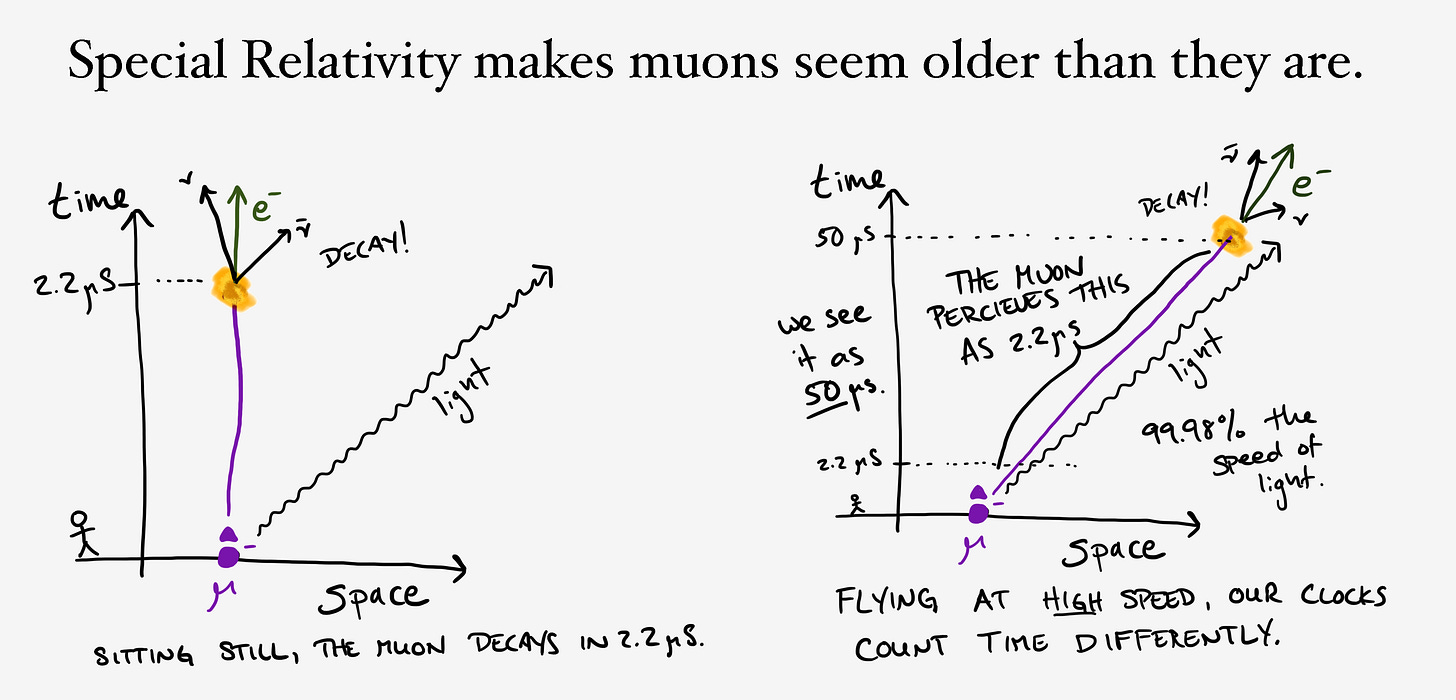
Typical energies for muons at sea level range from 1 GeV to 1 TeV, depending on the angle of incidence, for which muons appear to wait from 22 microseconds to 22 milliseconds before decaying to electrons. At least from out perspective.
In a muon collider, the muons in the beam will be accelerated to even higher speeds, speeds that would extend their apparent lifetime5 even further.
Synchrotron Radiation
As we’ve discussed, centripetal acceleration causes charged particles to radiate away their kinetic energy as light. The lighter the particle, the more susceptible to this energy loss they are.
Indeed, the rate of energy loss goes like one over the mass to the fourth power. Thus, the mass ratio of the muon to the electron suggests that the power radiated by muons is suppressed by a factor of 206^4! That’s 1.8 billion times less energy radiated.
Given the massive energy loss for electrons running in rings, you might wonder why we use circular accelerators at all. Wouldn’t it be more efficient to use a linear collider instead?
It depends. SLC at SLAC was linear. The proposed CLIC experiment - still under development - is also linear. The trouble with linear colliders is that the accelerated particles can only pass each accelerating RF cavity once on the way to their collision. Circular colliders can use them multiple times by passing them through the loop multiple times.
The LHC at CERN has such 16 acceleration points around its 27km ring, giving ample opportunities for particle acceleration.
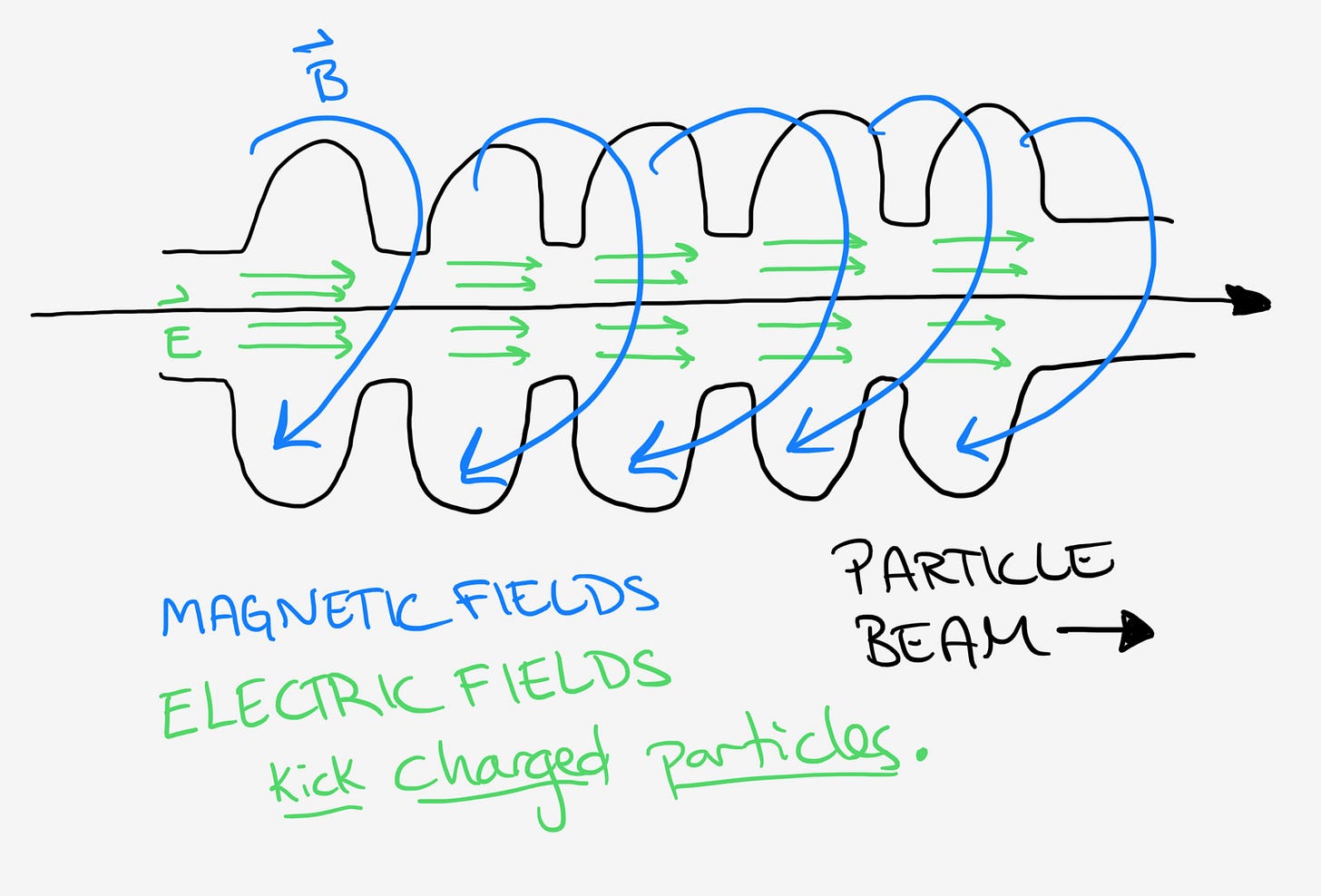
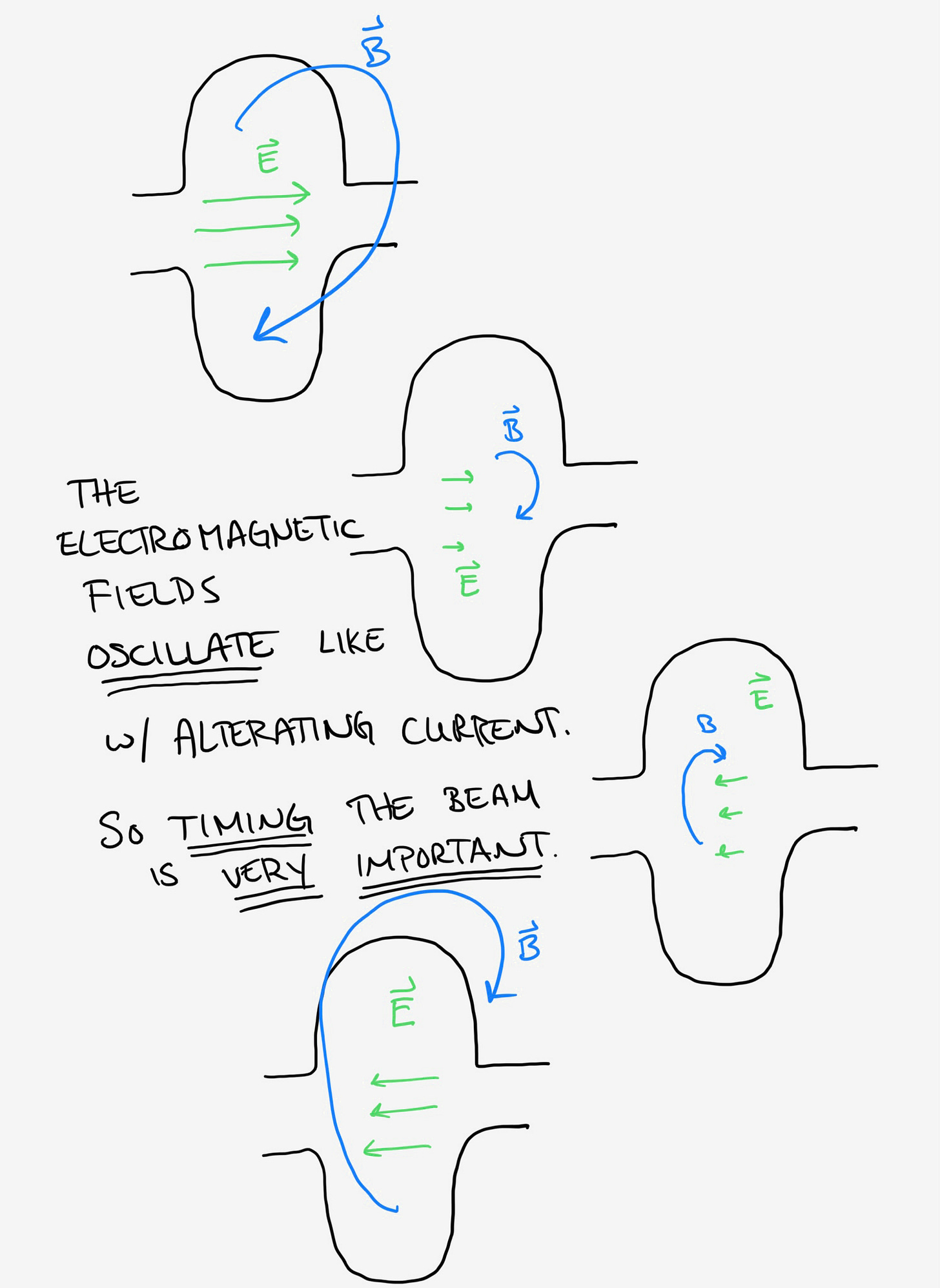
At the LEP experiment, which accelerated electrons and positrons in the very same tunnel in which the LHC now sits, it would take at least 9 orbits to get up to collision energy. Given that the tunnel is 27 km in circumference, the required linear tunnel size would be close to the distance from Geneva to Milan!
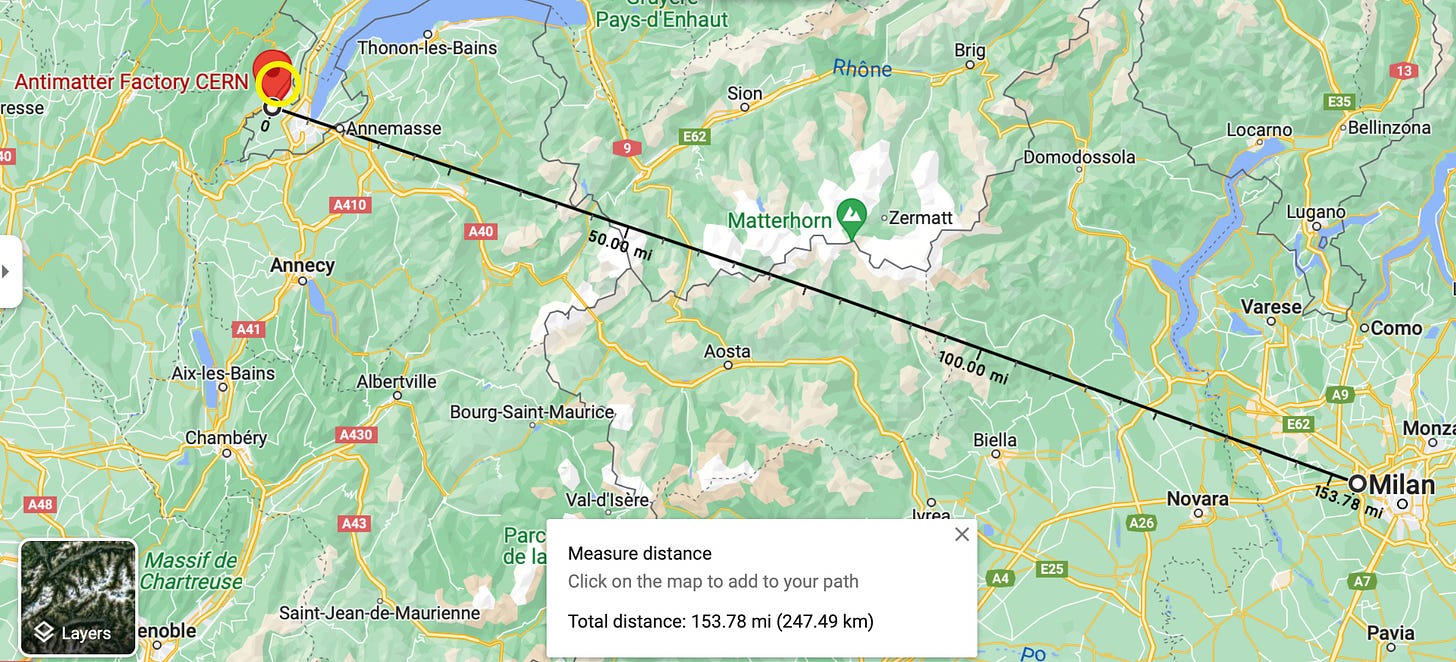
It’s just impractical.
In Summary
Hadron colliders achieve the highest energy collisions because they are more efficient than electron/positron colliders. These lepton colliders are built specifically to study the precise details of particle physics without all the mess hadrons make. Muon colliders win on both clarity of signal and efficiency of acceleration. The only trade-off is that muons are unstable particles.
Linear colliders could solve some of the efficiency issue, but they’re just not as effective. The CLIC experiment is proposing to study the physics of the Higgs Boson at a design energy that will scale from 380 GeV to 1 TeV and finally to 3 TeV.
The reach of a first generation muon collider is expected to be closer to 10 TeV, well over three times higher that CLIC! It would be comparable to the LHC in energy scale but without all the noise associated to hadron collisions. Because of this, it’s quite possible that new physics would be discovered in a muon collider.
So again. We should really build a muon collider.
More precisely, the muon’s half-life at rest is 2.2 microseconds, meaning a collection of muons - such as those in a beam - will decay by half each couple of microseconds! Fortunately, as we’ll see below, special relativity improves this performance by quite a bit
See also this early YouTube video we made.
There are other decays along this vein. Sometimes you’ll get a bonus electron-positron pair out of the decay. Very rarely, a bonus photon will come out. But these are all in addition to the electron and the neutrini.
Generically muons generated from cosmic ray impacts will have all kinds of different speeds. It just so happens that a lot of them are really, really fast. 99.98% of the speed of light is a fairly generic example.
In principle, although the muons need to be accelerated and brought up to that speed, which of course takes some time. This kind of technology - bringing those muons to life, then up to speed while keeping them collimated in usable beam - will be part of the fruits of building a muon collider.